
Downloads
Download




This work is licensed under a Creative Commons Attribution 4.0 International License.
Review
Emerging and Novel Therapeutic Treatments Targeting Mitochondrial-Endoplasmic Reticulum Contact Sites in Metabolic and Vascular Disorders
Richard M. Monaghan
The British Heart Foundation Centre of Research Excellence Manchester, Division of Cardiovascular Sciences, Faculty of Biology, Medicine, and Health, The University of Manchester, AV Hill Building, Oxford Road, Manchester, M13 9PN, UK;
richard.monaghan@manchester.ac.uk
Received: 10 April 2024; Revised: 5 May 2024; Accepted: 7 May 2024; Published: 6 June 2024
Abstract: Subcellular organellar contact sites, particularly those between mitochondria and the endoplasmic reticulum (MERCSs), play crucial roles in maintaining health. These specialized partitions facilitate vital communication between the organelles, regulating processes essential for cell function, including calcium balance, lipid biogenesis and transport, mitochondrial dynamics, and programmed cell death. Growing evidence shows that perturbation of MERCSs contributes significantly to various diseases, including neurodegenerative disorders like Alzheimer’s and Parkinson’s, metabolic issues, such as type 2 diabetes, heart conditions, and cancer. This review dives into this expanding field, exploring MERCSs as potential therapeutic targets. It provides a detailed overview of the proteins and processes that form and maintain MERCSs, highlighting how their disruption can lead to cellular dysfunction and disease. Additionally, it examines recent exciting breakthroughs in developing drugs and strategies that can manipulate MERCSs for clinical benefits. While challenges remain, this review emphasises the potential of MERCS-based therapies and outlines the critical research needed to move these treatments from the lab to the clinic.
Keywords:
MERCSs neurodegeneration calcium signaling lipid metabolism mitochondrial dynamics1. Introduction
Mitochondria, the powerhouses of the cell, are primarily responsible for generating adenosine triphosphate (ATP), the cell’s energy currency, through oxidative phosphorylation. Beyond energy production, mitochondria play vital roles in calcium signaling, regulation of programmed cell death (apoptosis), and the synthesis of lipids and heme. They are double membrane bound organelles whose dappled network of invaginations, or cristae, create a significantly large enough surface area to volume ratio, to enable the most efficient generation of the proton motive force, required for ATP generation [1]. The endoplasmic reticulum (ER) is a vast network of interconnected membranes forming sheets and tubules. The ER is essential for protein synthesis, folding, and quality control, ensuring only correctly folded proteins are transported. Additionally, the ER is a major site of lipid biosynthesis and plays a central role in calcium storage and signaling, which is crucial for numerous physiological cellular processes [2,3].
Mitochondrial-endoplasmic reticulum contact sites (MERCSs) are specialized zones where the outer mitochondrial and ER membranes come into close apposition (10‒30 nm), enabling direct communication between these vital organelles [4]. Structurally, MERCSs rely on a variety of tethering complexes that physically bridge the gap. One prominent example is the VDAC1-IP3R-GRP75 (Voltage-Dependent Anion Channel 1-Inositol Triphosphate Receptor-Glucose-Regulated Protein 75) complex, where VDAC1 resides on the mitochondria, IP3R on the ER, and GRP75 acts as the tethering protein between the two outer organellar membranes. This complex regulates transport of calcium and other metabolites between the two organelles [5]. Further research on this tethering network has identified several other associated proteins including the Translocase of the Outer Mitochondrial Membrane 70 (TOMM70) [6], transglutaminase 2 [7], thymocyte-expressed positive selection-associated protein 1 [8], Parkinson disease protein 7 (DJ-1), and pyruvate dehydrogenase kinase 4 (PDK4) [9]. Adding further regulatory complexity to the homeostatic signaling role of MERCSs [10].
Other protein complexes have been shown to act as bridges between the two organelles. For example, Mitofusins 1 and 2 (MFN1 and 2), also play a tethering role at MERCSs. Localization of MFNs on proximal sites of both mitochondrial and ER membranes and protein-protein interactions facilitate their tethering. Intriguingly, full length MFN2 present on the mitochondrial outer membrane, has been shown to interact with an MFN2 splice variant that encodes a shorter form of the protein, ERMIT2 (ER Mitofusin 2 tether), on the ER membrane, enabling interaction between the two organelles [11,12]. Presenilins (PSENs) are transmembrane proteases with established roles regulating lipid, including cholesterol/phospholipids/sphingolipids, and calcium homeostasis at the mitochondria and ER [13]. PSEN1 and 2 are both found enriched in MERCSs, and double knockout of both proteins in mice, and cell lines derived from these animals, display alterations to mitochondrial morphology, affiliated with impaired oxygen consumption and utilisation, usually used for ATP production, and hence spare respiratory capacity. Decreased expression of oxidative phosphorylation complex subunits and irregular mitochondrial cristae structure were also observed compared to wildtype. However, compensatory mechanisms, through an increase in glycolytic flux, identified no alterations to the ATP/ADP ratio or, crucially a key feature of mitochondria, their membrane potential [14]. PSEN2 was identified as the main facilitator of this mechanism, and interactions with MFN2 were also demonstrated, suggesting these membrane proteins are critical for MERCS function [15]. The mitochondria-associated ubiquitin ligase, MARCHF5 (Membrane Associated Ring-CH-Type Finger 5, also known as MITOL), and glycoprotein 78 (GP78), also a ubiquitin ligase, work cyclically to regulate MFN2 stability, through which they are able to regulate MERCSs, and lipid and protein homeostasis [16–18].
The interaction between VAPB (Vesicle-Associated membrane protein-associated Protein B) and PTPIP51 (Protein Tyrosine Phosphatase-Interacting Protein 51) represents another important connection for MERCSs and plays a role in their regulation of calcium signaling [19,20]. Similarly, another central MERCS tethering complex is mediated by BAP31 (B cell Receptor Associated Protein 31), FIS1 (mitochondrial FISsion protein 1), and DRP1 (mitochondrial Dynamin-Related Protein 1), during the regulation of apoptosis, and mitochondrial fusion and fission [21,22]. The Mitochondrial Rho GTPase (MIRO) has also shown to act as a MERCSs conduit in coordination with VPS13D (Vacuolar Protein Sorting 13 homologue D) [23]. BAP31 also interacts with another protein identified in MERCSs, TOMM40, ensuring the proper function of complex I of the electron respiratory chain that initiates generation of the proton motive force that drives ATP production, as previously mentioned, the major energy currency of the cell [22].
Briefly, as they are applicable to the associated pathologies discussed in the main text, the following proteins are also known to be MERCSs components/regulators: Synaptojanin 2 Binding Protein (SYNJ2BP) and Ribosome Binding Protein 1 (RRBP1) have been shown to be tethering partners [24]; Foetal and Adult Testis Expressed 1 (FATE1) plays a role in the coupling of the two organelles [25]; CDGSH Iron Sulphur Domain 2 (CISD2) is a MERCSs component that regulates both lipid transfer and metabolism, and calcium homeostasis [26,27]; ATPase family AAA Domain containing 3A (ATAD3A) plays a scaffolding role for mitochondrial structure but has also been associated with MERCSs [28]; FUN14 Domain-Containing protein 1 (FUNDC1) is a mitochondrial membrane protein that plays key roles in mitophagy (the turnover of mitochondria) and is associated with hypoxia-related IP3R binding [29]; finally, Cytoskeleton-Associated Protein 4 (CKAP4) regulates mitochondrial and ER functions, including ER morphology, whilst also acting as a tethering partner with VDAC2 [30].
Through the tethering complexes above and a rapidly growing field of research into novel MERCSs constituents, it has been established that they act as hotspots for cellular calcium homeostasis, allowing for fine-tuning of mitochondrial metabolism and cell survival signaling [10]. They also facilitate the transfer and metabolism of lipids, such as phosphatidylcholine and cholesterol, the former of which is a precursor for many mitochondrial lipid-metabolising biosynthetic pathways, the latter a crucial, regulated constituent of subcellular organelle membranes. Thus, regulation of lipids by MERCSs is crucial for membrane biogenesis and lipid-associated signaling pathways. MERCSs have an essential role influencing mitochondrial fission and fusion, processes that are critical for maintaining cellular health and function [31]; autophagic responses, where they can serve as platforms for its initiation, a process whereby the cell degrades and recycles damaged components [32]; and a key cellular role triggered through dysregulation of calcium signaling, and other stressors, during induction of apoptosis (Figure 1) [33]. An array of signaling pathways have been shown to regulate MERCS components and function, through posttranslational modifications, that could also be targeted therapeutically to remedy MERCSs-associated pathophysiology [34].
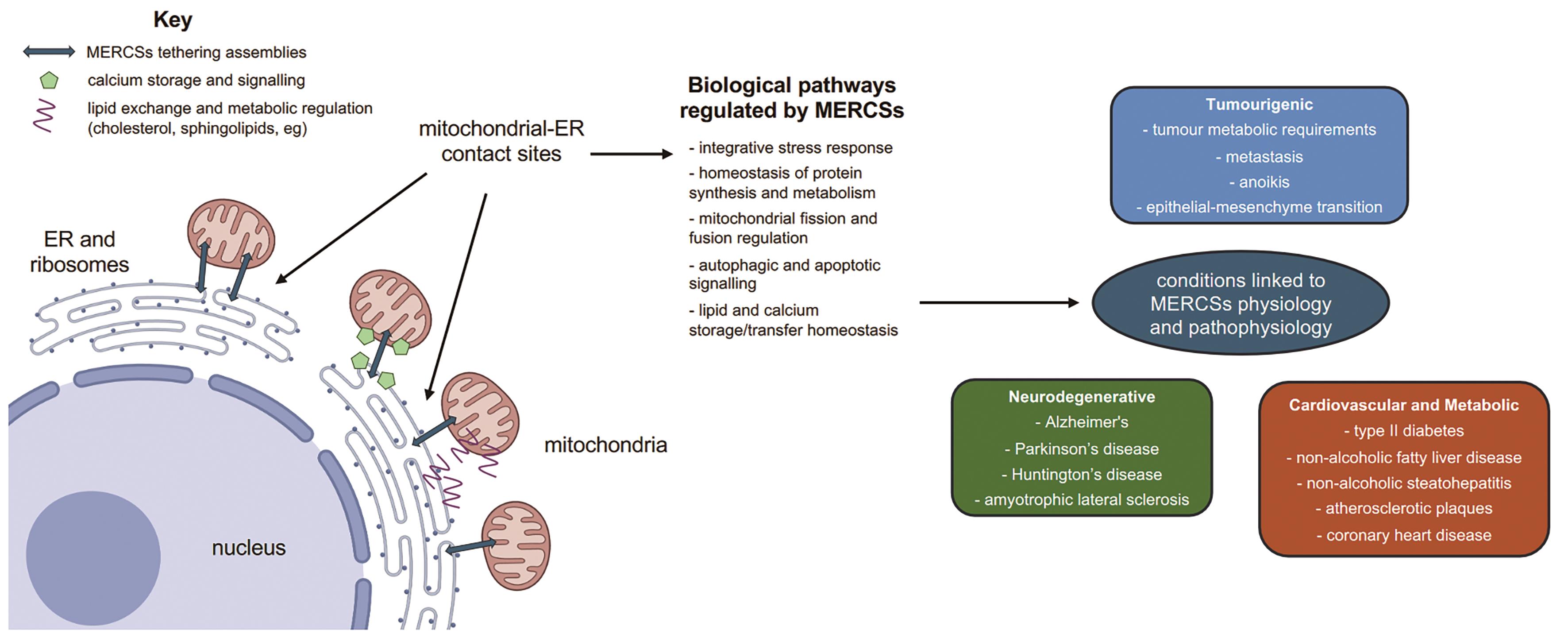
Figure 1 Schematic of the key functions of MERCSs physiology and pathophysiology. Subcellular diagram of MERCSs, list of their biological functions, and human disease conditions associated with their interrupted and/or dysfunctional states. For references see main text.
MERCSs are responsive to various cellular conditions, including calcium levels, energy demands, and cellular stress, including disrupted ribosomal or mitochondrial proteostatic dysfunction [35]. These factors trigger the recruitment and assembly of specific proteins and lipids to the contact sites, modulating MERCS function to meet the evolving needs of the cell (Figure 1) [36,37]. There are almost certainly more mitochondrial-ER tethering proteins yet to be discovered, and understanding their roles, and those of already established bridging centers, in the organisation of highly dynamic MERCS complexes, how they change during development, in different cell types with distinctive metabolic demands, and in pathophysiological processes, are research endeavours that are becoming profuse.
2. Disease Pathogenesis-associated with MERCSs
2.1. Neurodegenerative Diseases
Dysfunction of MERCSs plays a significant role in the development of Alzheimer’s disease (AD) [38]. MERCSs are crucial for regulating calcium transfer between the ER and mitochondria; their disruption in AD leads to mitochondrial calcium overload, promoting oxidative damage and neuronal cell death pathways [39, 40]. Additionally, altered lipid metabolism at MERCSs compromises neuronal membrane integrity and may even influence the production of toxic amyloid-beta (Aβ) peptides [41]. MERCS dysfunction also contributes to ER stress and excessive mitochondrial fragmentation, further exacerbating neuronal dysfunction and energy deficits [42]. Recently, an alternative link between MERCSs and AD has been proposed, where the C99 intermediary, from which the Aβ peptides are cleaved, is the pathogenic driver of disease. C99 regulates cholesterol transport and its intussusception with membranes of subcellular organelles, is localises to MERCSs. It has been postulated that it is in fact increased levels of C99 at MERCSs concomitant with increased cholesterol transport to the membranes of organelles that is the pathogenic mechanism of AD, marking it as a disorder of lipid transport, not protein aggregation [43]. Whichever hypothesis is correct, MERCS-related abnormalities, in particular PSENs, can contribute to the cognitive decline and neurodegeneration characteristic of AD, highlighting the potential of targeting MERCSs as a therapeutic strategy [44,45].
Aberrant activity of MERCSs contributes significantly to the pathology of Parkinson’s disease (PD) [46]. In PD, MERCSs become disrupted, hindering the crucial regulation of calcium exchange between the ER and mitochondria [47]. This disruption leads to mitochondrial calcium excess, resulting in increased production of damaging reactive oxygen species (ROS), compromised energy production (ATP synthesis), and the activation of cell death pathways [48]. Additionally, overwhelmed by calcium dysregulation and other MERCS-related alterations, the ER experiences stress. This promotes the misfolding of proteins, including alpha-synuclein – a key protein that forms toxic aggregates in PD [49]. Furthermore, MERCSs dysfunction is linked to mutations in proteins such as PINK1 (PTEN-induced kinase 1) and Parkin, which usually interact with MERCSs to facilitate the removal of damaged mitochondria (mitophagy). Impaired mitophagy leads to the accumulation of dysfunctional mitochondria, further exacerbating cellular stress. MERCS dysfunction and the resulting cellular damage can also trigger inflammatory responses within the brain, contributing to a damaging cycle that worsens neurodegeneration [46].
In Huntington’s disease (HD), it has been shown that disrupted MERCSs impair calcium regulation, leading to mitochondrial dysfunction and ER stress [50,51]. The mutant huntingtin protein further exacerbates the problem by interacting with MERCSs, promoting excessive mitochondrial fragmentation. These combined effects contribute to the neuronal dysfunction and cell death characteristic of HD [50,52].
Improper function of MERCSs plays a significant role in the development of Amyotrophic Lateral Sclerosis (ALS) [53]. ALS is the most common form of motor neuron disease; it is a perturbing neurodegenerative condition that presently has no cure. Disrupted MERCSs in the central nervous system that usually regulate mitochondrial metabolism and act as gatekeepers to autophagy, lead to ALS progression. Impaired calcium regulation, resulting in mitochondrial dysfunction and ER stress also play a role in disease pathogenesis. These disruptions promote the misfolding and aggregation of proteins implicated in ALS, such as TDP-43 (tar DNA-binding protein 43) and SOD1 (superoxide dismutase 1). Additionally, mutations in genes associated with ALS can disrupt lipid metabolism at MERCSs, affecting membrane integrity and neuronal function [54]. In HD MERCS dysfunction may hinder the process of mitophagy, contributing to the accumulation of unhealthy mitochondria. The most significant MERCS constituents implicated in ALS are VAPB and VCP (Valosin Containing Protein). VCP is a ubiquitin-specific ER chaperone, and in coordination with the VAPB tethering complex, is responsible for maintaining mitochondrial metabolic output and preventing harmful metabolic ROS by-products. Mutations found in upstream regulators of these two complexes have been associated with ALS progression, for example, FUS and C9orf72 [55]. These combined effects of MERCS dysfunction severely compromise the health of motor neurons, ultimately leading to the degeneration and paralysis characteristics of ALS [56].
A CRISPR screening approach, for genes whose decrease in expression increased mitochondrial-ER proximity, recently identified two novel MERCSs components, Guided Entry of Tail-anchored proteins factor 4 (GET4) and BCL2-associated Athanogene 6 (BAG6). Both proteins were shown to interact with IP3R and GRP75 and decreasing GET4 enhanced mitochondrial calcium uptake from the ER, and improved respiration. Remarkably, in a fly model of AD it was also shown that loss of GET4 improved motor ability, increased lifespan, and prevented neurodegeneration, indicating GET4 as a potential therapeutic target for neurovascular disease [57].
2.2. Cardiovascular and Metabolic Conditions
Inappropriate activity of MERCSs contributes to the development of Type 2 Diabetes (T2D) [58]. MERCSs play a role in regulating insulin signaling, and their disruption in T2D can lead to ER stress and altered lipid metabolism, both of which impair insulin action [59]. Additionally, MERCS dysfunction compromises mitochondrial function, particularly in insulin-producing pancreatic beta cells, hindering their ability to secrete sufficient insulin. The combined effects of ER stress, mitochondrial damage, and MERCS dysfunction also contribute to chronic inflammation, a key factor in insulin resistance [60,61]. These consequences ultimately lead to the insulin resistance, impaired insulin secretion, and overall metabolic imbalance characteristic of T2D [62].
Defective MERCSs play a significant role in obesity and its associated health complications. The excess nutrient intake and increased metabolic demand associated with obesity can trigger ER stress, disrupting MERCS function [63]. This disruption impairs lipid storage within fat cells, alters lipid metabolism, and compromises mitochondrial energy production. It has been demonstrated that in the adipocytes of obese mice mitochondria are severely disordered, by a process mediated by RalA (RAS Like Proto-Oncogene A) mechanistically causing inhibitory phosphorylation of Drp1. Conditional deletion of RalA in white adipocytes prevented mitochondrial fragmentation, and decreased weight gain when fed a high calorie diet by stimulating fatty acid oxidation, indicating that targeting the MERCSs fission protein Drp1 could represent a clinical option for individuals who are overweight [64]. Additionally, MERCS-related ER stress, mitochondrial dysfunction, and altered lipid handling contribute to chronic inflammation. These combined effects worsen metabolic dysfunction, promote further fat accumulation, and increase the risk of obesity-related complications such as T2D, cardiovascular disease, and Non-Alcoholic Fatty Liver Disease (NAFLD) [64–66].
Dysfunction of MERCSs displays a significant role in the development and progression of NAFLD [67]. MERCSs are crucial for regulating lipid metabolism, and their disruption in NAFLD leads to increased production and decreased removal of harmful fats within the liver. This, coupled with metabolic stress, triggers ER stress, which further impairs MERCS function. Additionally, MERCS dysfunction compromises mitochondrial energy production and promotes oxidative damage [68,69]. The resulting lipid accumulation, ER stress, mitochondrial dysfunction, and inflammation drive the liver damage, scarring, and progression from simple NAFLD to the more severe form, non-alcoholic steatohepatitis (NASH) [70–72].
Incorrect function of MERCSs can contribute to the development and progression of cardiovascular disease (CVD) [73,74]. MERCSs are involved in lipid metabolism, and their disruption can lead to the build-up of harmful fats and promote inflammation within blood vessels, accelerating the formation of atherosclerotic plaques [75,76]. Additionally, MERCS dysfunction impairs calcium signaling and energy production in both heart muscle cells and the cells lining blood vessels (endothelial cells). This impairment compromises heart function, weakens blood vessel health, and increases the risk of complications like heart attacks, strokes, and heart failure [77,78].
2.3. Tumourigenesis
Abnormal function of MERCSs plays a significant role in the growth and survival of cancer cells. MERCSs are often altered in cancer, leading to changes in calcium signaling that promote uncontrolled cell division and resistance to cell death [79]. Additionally, MERCS dysfunction contributes to the metabolic reprogramming of cancer cells, supporting the increased energy and lipid production necessary for rapid growth [80]. Further, MERCS alterations can help cancer cells evade programmed cell death (apoptosis) and enhance their ability to migrate and invade other tissues (metastasis) [81]. These effects make MERCSs a potential target for developing new cancer therapies aimed at disrupting tumour growth, increasing sensitivity to treatment, and preventing spread [82]. CISD2 has known roles in tumourigenesis and other age-related diseases identifying it as a promising clinical target [26,83,84]. CKAP4 has also been associated with cancer and could act as a biomarker for specific malignancies [85].
The promotion of metastasis by MERCSs, is enabled by their dysfunction, helping cancer to spread. MERCSs regulate calcium signaling, and alterations in cancer cells lead to changes in cell migration, invasion, and resistance to cell death during detachment (anoikis) [86]. Additionally, MERCS dysfunction can contribute to the breakdown of cell adhesion and changes associated with epithelial-mesenchymal transition (EMT), further enhancing a cancer cell’s metastatic potential. Targeting MERCSs might offer a new approach to hinder metastasis by disrupting the processes that allow cancer cells to invade, survive in circulation, and colonise distant sites [87].
It is important to note that the exact mechanisms linking MERCS dysfunction to disease are complex and vary between diseases. Research in this area of fundamental biology is rapidly evolving, the protein constituents of these tethers, how these change between cell types and developmental stages, has not been fully elucidated, but this will lead to a greater understanding of the potential therapeutic targets MERCSs represent for multiple pathologies [88].
3. Strategies for Targeting MERCSs
3.1. Modulating Protein-Protein Interactions at MERCSs
A promising strategy for targeting MERCSs involves the use of small molecules or other compounds that can directly interfere with protein-protein interactions (PPIs) within these crucial contact sites [89]. Researchers have identified compounds that bind to specific MERCS tethering proteins, preventing them from interacting [41]. For instance, molecules that disrupt the interaction between VAPB, on the ER and, PTPIP51, on mitochondria, have shown promise in preclinical studies [90]. Peptide-based inhibitors, short peptide sequences mimicking the binding domains of MERCS proteins can compete with the native proteins, effectively disrupting the formation of the complex [91].
Targeting MERCSs, in particular GRP75, following myocardial infarction, when calcium signaling is greatly perturbed and mitochondrial and ER stress pathways are activated, has been shown in rats as a potential therapy to reduce these detrimental side effects, without altering homeostatic hypoxic signaling, and preventing further damage to the heart [92]. It has also been demonstrated that FUNDC1 deficiency perturbs mitochondrial quality control mechanisms and exacerbates high caloric diet-induced obesity and concomitant metabolic syndrome, indicating another protein target, for tackling metabolism-related MERCSs disorders [93].
NASH is linked to metabolic dysfunction and can progress to liver cancer. GP78 appears to play a protective role in disease progression. Mice without Gp78 develop age-related obesity and NASH-like symptoms, including fatty liver, inflammation, scarring, and tumours. This decline is triggered by stress signaling within the cell and increased fat production. Importantly, in human liver cancer samples, lower GP78 levels correlate with more severe disease. This suggests GP78 could be a pivotal therapeutic target, and that it is crucial for maintaining liver health and may act as a tumour suppressor [94].
Urolithin A is produced by the gut microbiome and acts as a naturally occurring chemical that could be utilised in treatment regimens focused on anti-inflammatory, ROS reduction, longer healthspan, and prevention of muscle atrophy. Although its mechanism of action is not clear, it reduces autophagy and promotes mitochondrial biogenesis, demarcating urolithin A as a potential MERCS therapeutic strategy [95]. Another promising small molecule treatment for diseases associated with chronic low-grade inflammation, often associated with dysfunction of MERCSs, is l-sulforaphane. Cruciferous vegetables contain high levels of this antioxidant anti-inflammatory agent, suggesting moderate lifestyle/diet alterations could have longer term beneficial effects through their role in modifying MERCS biology [96].
The BH4 domain of the antiapoptotic protein BCL2 (B Cell Lymphoma 2) is what distinguishes it compared to other BCL2-family members, which actively drive programmed cell death. Interestingly, a TAT (cell penetrating peptide conjugate)-BH4 fusion peptide has been shown to inhibit apoptosis and improve survival in models of disease caused by enhanced apoptosis. Hence, the BH4 domain alone has antiapoptotic properties. It has been reported that the BH4 domain mediates interaction of Bcl2 with IP3R on the ER. Through binding the regulatory and tethering domains of IP3R the BH4 peptide is able to inhibit IP3R-dependent calcium release from the mitochondria and thus, prevent activation of apoptosis. This targeted feature of the BH4 domain peptide makes it an attractive prospect for treatment of multiple MERCS-associated conditions [97].
3.2. Stabilising MERCS
Small-molecule stabilisers offer a promising therapeutic approach for targeting MERCSs. These molecules are designed to bind at the precise point where key MERCS proteins interact. By acting like a support, they can strengthen the connection between these proteins, enhance their affinity for each other, and prevent them from disassociating [35]. This stabilisation is particularly beneficial in diseases where MERCS function is compromised, as it can help restore this crucial communication between mitochondria and the endoplasmic reticulum. The targeted nature of small-molecule stabilisers, along with the widespread importance of MERCs in cellular processes, suggests their potential therapeutic use for a diverse range of diseases [37,98].
Recently the role apelins have maintaining MERCS stability has been therapeutically investigated; apelin-13 has already shown promise treating ischaemia-associated disorders, diabetic complications, CVD, and neurodegenerative disease. Apelin-13 is an endogenous ligand for the G-protein coupled receptor angiotensin II protein J (AT2R), with downstream signaling effects on mitochondrial and ER functioning. In diabetic-associated hearing loss, treatment of cochlear hair cells following culture in high glucose media, with apelin-13 elevated mitochondrial membrane potential and ATP production, whilst decreasing damaging oxidative by-products. The levels of ER-associated stress proteins were also decreased, but treatment with the ER stress agonist tunicamycin abrogated these effects. This revealed the protective role of apelin‑13 in restructuring mitochondrial functional impairment in cochlear hair cells by inhibiting ER stress following high glucose treatment [99,100].
Molecular glues represent an innovative strategy for stabilising weakened MERCS complexes. These specialised molecules have the unique ability to act as bridges, simultaneously binding to two distinct proteins and promoting their association. Unlike traditional drugs that often target a single protein, molecular glues can facilitate the formation or increase the stability of protein-protein complexes. In the context of MERCSs, a molecular glue could be designed to bind specific sites on both an ER-resident protein and its mitochondrial counterpart, strengthening their interaction and restoring the integrity of a compromised MERCS [101].
Ageing and heart failure are characterised by decreased mitochondrial stability and increased mitophagy, with excessive ketone body production also observed. Targeting this pathway has been shown to facilitate mitochondrial structural integrity and decrease mitochondrial turnover. The TAT-MP1(Gly) cell permeable peptide was able to promote MFN2 recruitment to impaired and mitochondria and increase mitochondrial fusion over fission [102]. Another TAT fusion peptide, that mimics the phosphorylated region of phospholamban, an endogenous inhibitor of a key ER calcium release channel. Through phosphomimectic mutations of the two phosphorylated residues in a nine amino acid sequence of phospholamban, its inhibitory phosphatase is prevented from dephosphorylating the endogenous protein thus preventing inappropriate calcium release from the ER, making it an appealing MERCSs target [103].
3.3. Targeting the Transfer of Lipids to Affect MERCSs Function
Regulating lipid transfer offers an indirect strategy for influencing MERCS function. Since lipids serve as structural components of MERCSs and play vital roles as signaling molecules, compounds that affect lipid synthesis or transport can have downstream effects on MERCS stability and activity [104]. For instance, altering the levels of specific phospholipids or cholesterol may impact the formation of MERCSs, while modulating lipid signaling pathways could influence MERCS-associated protein complexes. Additionally, preventing lipid overload and reducing ER stress can indirectly promote healthy MERCS function [105]. While careful consideration of specificity and potential side effects is needed, manipulating lipid metabolism presents a promising avenue for indirectly targeting MERCSs in the treatment of related diseases [106].
Iron accumulation is often observed in alcoholic liver disease (ALD), suggesting a potential role for ferroptosis, a type of programmed cell death dependent on iron and characterised by the accumulation of lipid peroxides, in its development. Quercetin, a natural compound, may have protective effects against ferroptosis in ALD. This protection may be linked to its influence on MERCSs and their regulation by an unfolded protein response mediator, the kinase PERK (PKR-like ER Kinase). Interestingly, PERK’s structural role, rather than its typical enzymatic activity, seems to be important for MERCS formation and ferroptosis in the context of alcohol exposure. These findings suggest that quercetin could be a potential therapeutic agent for mitigating alcohol-induced liver injury by targeting ferroptosis [107].
3.4. Modulation of Calcium Signaling
MERCSs serve as hubs for calcium signaling between mitochondria and the ER. A promising therapeutic approach involves modulating the calcium channels and transporters specifically located at these contact sites [108]. Strategies include the use of agonists and antagonists for IP3Rs (the ER’s calcium release channels), inhibitors for VDACs (mitochondrial outer membrane channels), as well as molecules targeting the MCU (Mitochondrial Calcium Uptake channel) [109,110]. Additionally, influencing mitochondrial calcium efflux mechanisms, such as activating the NCLX (mitochondrial sodium/calcium exchanger), provides an alternative avenue for intervention [111]. Importantly, the development of such therapeutics requires careful attention to specificity, aiming to precisely target MERCS-based calcium mechanisms, along with an understanding of the delicate balance necessary for healthy calcium signaling [112].
Activating Transcription Factor 6 (ATF6) has been shown to regulate mitochondrial and ER calcium homeostasis. ATF6 is part of the signaling pathways that communicate the unfolded protein response from the ER to the nucleus, but also affects lifespan through MERCSs calcium homeostatic regulation, and changes to related apoptotic signaling pathways [113,114]. In worms atf-6 depletion increases lifespan through altering calcium release through itr-1 (orthologue of IP3R) and mitochondrial calcium disorders of uptake through the mcu-1. Indicating targeting ATF6 as a potential clinical goal for MERCSs-associated calcium homeostasis [115]. PSEN2 through its regulation of calcium signaling and mitochondrial function also represents another potential clinical target for modulating MERCSs biology, particularly in neurodegenerative diseases [116].
ALD involves the harmful build-up of calcium within liver cell mitochondria, but the exact trigger for this overload has been unclear. Alcohol exposure leads to an abnormal increase in PDK4 activity, which then promotes excessive calcium channelling from the ER into the mitochondria. This calcium overload disrupts mitochondrial function, contributing to liver damage. It has been shown in human patients with ALD that this is indeed the case, opening new doors to potential therapeutic strategies focused on regulating PDK4 activity or preventing the formation of calcium-channelling [117].
Tumour suppressor protein, p53, plays a role in cell death beyond its known actions in the nucleus. In the cytoplasm, p53 interacts with the ER and mitochondria, influencing calcium flow between these compartments. This calcium transfer is crucial for triggering apoptosis. When activated by stress, p53 accumulates at these contact points and alters calcium balance in the ER, leading to increased calcium transfer to the mitochondria. This overload disrupts the mitochondria and ultimately leads to cell death. Indicating a new, non-nuclear function of p53 in regulating calcium-dependent cell death, and a potential target for clinical intervention in MERCSs pathophysiology [118].
In a screen for modulators of mitochondrial dysfunction, in the context of neurodegenerative, ageing, and metabolic dysfunction. Luteolin was identified as increasing mitochondrial activity in neurons, whilst not increasing mitochondrial size. Intriguingly, luteolin was found to increase the number of mitochondrial-ER contacts with a concomitant beneficial effect on calcium exchange, and mitochondrial pyruvate dehydrogenase, and respiratory chain complex I and II function. This indicates the potential of luteolin treatment for a wide range of MERCS-associated conditions [119,120].
3.5. Directly Targeting Processes that Regulate Mitochondrial Dynamics
Targeting mitochondrial dynamics, the balance between fission (facilitated by proteins like DRP1 and FIS1) and fusion (mediated by proteins like MFN1, MFN2, and Optic Atrophy 1 (OPA1)) is a potential therapeutic strategy with implications for MERCS function [121]. Drugs like mdivi-1 (a cell permeable quinazolinone) and dynasore (a noncompetitive inhibitor of the dynamin GTPase), inhibit fission, aiming to reduce excessive fragmentation, while fusion promoters like Mitochondrial Fusion Promoter M1, a hydrazone compound, enhance MFN2 activity [100,122]. While these drugs primarily target mitochondrial shape, they could indirectly influence MERCSs by altering the number of interaction sites.
Interestingly, MFN2 is also found in MERCSs, suggesting a potential direct link between mitochondrial dynamics and MERCS regulation. Research into this area holds promise for diseases where both mitochondrial dynamics and MERCSs are disrupted, but challenges remain in developing highly specific drugs (like P110-TAT) and fully understanding the complex interplay between these proteins and cellular processes [123].
ATAD3A mutations have been identified in individuals with neurological symptoms, often causing death at a young age. The vast majority of these mutations are loss of function or dominant negative genetic variants. ATAD3A’s pivotal role in MERCSs biology, in particular in regulation of mitochondrial dynamics, make it a key target for therapeutic interventions, in which stabilisation of mitochondrial fission or fusion processes are required [124]. Metformin, an established diabetic treatment, may also be beneficial for other MERCSs related disorders. For example, in patients with type II diabetes, metformin treatment was shown to decrease ROS, increase expression of mitochondrial respiratory chain subunits, reduce mitophagy and enhance activation of a key upstream regulator of mitochondrial physiology, AMPK (adenosine monophosphate-activated kinase) [125].
Inflammation and the presence of M1 macrophages play a key role in the development of atherosclerosis (plaque build-up within arteries). DRP1, may be a promising target for reducing this harmful inflammation. Mdivi-1 DRP1 inhibition reduces plaque formation, decreases M1 macrophage presence, and lowers inflammation markers. Investigations showed that oxidised LDL (low density lipoprotein, or ‘bad’ cholesterol) fuels M1 activation and mitochondrial damage, and compounds that protect mitochondria also decreased inflammation. Similarly, Mdivi-1 appears to work by reducing M1 macrophage formation. These findings suggest that targeting DRP1-dependent mitochondrial breakdown could offer a potential new treatment strategy for combating atherosclerosis [100].
Antioxidant treatment for conditions in which mitochondrial and/or ER ROS production is above physiological levels are a promising avenue for therapeutic interventions. For example, trolox, a water-soluble analogue of vitamin E, has been shown to repair mitochondrial functioning, calcium signaling, and membrane potential in a model of human complex I deficiency [126]. Chemical modulators of dynein signaling have also shown promise in modulating these structural regulators of mitochondrial subcellular biology. Dynarrestin for example, is a reversible cytoplasmic dynein inhibitor that has shown potential as a therapeutic approach to tumourigenesis [127].
Mitochondria play a critical part in a cell’s capacity to adjust to ever changing microenvironments, and dysfunction of these responses has been shown to lead to cancer. Identifying novel biomarkers that arise when these processes are altered and the use of small molecule inhibitors to actively target their dysfunction is vital scientific discovery [128].
4. Challenges and Future Directions
4.1. Ensuring Specificity
Designing highly specific compounds that target only the desired MERCS proteins is crucial to minimise off-target effects in therapeutic development. MERCSs exist within complex cellular networks, and proteins associated with them often have roles beyond just MERCS regulation. Nonspecific targeting could disrupt these networks and vital cellular functions, leading to unintended side effects [129]. Strategies like detailed structural studies of MERCS proteins, targeted drug delivery mechanisms, and rational drug design can enhance specificity. However, challenges remain due to the limited structural information on some MERCS components and their dynamic nature. Ongoing research into MERCS structure and function, coupled with advancements in drug design, is essential to overcome these challenges and develop highly specific therapies that minimise off-target effects [130].
4.2. Delivery of Therapeutics
Strategies to effectively deliver MERCS-targeting compounds intracellularly and specifically to MCSs are needed to maximise their therapeutic potential. Since MERCSs reside inside cells, delivery systems must facilitate drug entry across the cell membrane and ideally concentrate the drug at MERCS locations [131]. Approaches like nanoparticles, liposomes, cell-penetrating peptides (CPPs, for example TAT)), or linking drugs to molecules that recognise MERCS components can enhance targeted delivery. However, challenges remain in ensuring the stability and biocompatibility of delivery systems while achieving sufficient drug concentration specifically at MERCSs. Ongoing research into optimising these delivery strategies is crucial for the successful translation of MERCS-targeted therapies into clinical practice [132]. MitoQ is a god example of a targeted therapeutic approach whereby antioxidant therapy is directed towards mitochondria by virtue of a specialised localisation mechanism, that could alleviate dysfunctional ROS generating MERCSs [133].
4.3. Combination Therapies
Combining MERCS-targeting drugs with existing treatment modalities holds promise for enhancing therapeutic outcomes in various diseases. Since these diseases often involve complex mechanisms, targeting MERCSs alongside other disease pathways could provide a multi-pronged and potentially synergistic approach. For example, in neurodegenerative diseases, MERCS-targeting drugs might be combined with antioxidants or anti-inflammatory therapies, while in cancer, they could increase the effectiveness of chemotherapy or immunotherapy [134]. Strategic combinations might also improve metabolic outcomes in conditions like diabetes or high cholesterol. However, careful research is needed to identify the most effective combinations, understand potential drug interactions, and manage the possible increased risk of side effects with combination therapy.
4.4. Translational Approaches
Translational research, including pre-clinical models and clinical trials, is crucial for validating the efficacy of MERCS-targeted therapies. Pre-clinical models allow for initial safety and efficacy testing, as well as in-depth analysis of how these therapies work. Clinical trials are essential to assess the drug’s performance in humans, confirm its effectiveness, and evaluate its risk-benefit profile [135]. Challenges include creating accurate pre-clinical models that mimic MERCSs in human disease and effectively translating animal study results into human outcomes. Optimising both pre-clinical models and clinical trial design is key for successfully moving MERCS-targeted therapies from the lab to the clinic to ultimately improve patient outcomes [136].
5. Conclusion
The intricate network of MERCSs in all cell types of the body offers a treasure trove of potential targets for the development of novel therapeutic strategies. The link between MERCSs disconnections and ageing, concomitant with age-related diseases, raises the possibilities of treating a broad range of human pathologies. While challenges remain in achieving specificity and optimising targeted drug delivery, the pace of recent discoveries highlights the immense promise of this field. See Table 1 for details regarding the range of different methods used with MERCS-targeting therapies. As our understanding of the molecular players and regulatory mechanisms at MERCSs grows, so too does our ability to design precise modulators of these crucial communication hubs. Further research and advancements in translational science hold the key to harnessing the therapeutic potential of MERCSs, paving the way for transformative treatments for a wide range of diseases, particularly those linked to ageing, in treating mitochondrial-ER tethering dysfunction.
Therapeutic Strategy | Biomolecular Pathway | Potential Therapies | References |
---|---|---|---|
Modulating MERCSs protein-protein interactions | VAPB, PTPIP51, GRP75, FUNDC1, GP78, IP3R, BCL2 | small molecule/peptide-based inhibitors, urolithin A, l-sulforaphane, TAT-BH4, diet | [89–97] |
Modulating MERCSs stability | MFN2, AT2R, phospholamban | TAT-MP1(Gly), apelin-13, molecular glues, TAT-phospholamban phosphomimetic | [35,37,98–103] |
Targeting lipid homeostasis to affect MERCS function | cholesterol levels in subcellular organelle membranes, lipid biosynthetic pathways, phospho/sphingolipids | PERK modulators, quercetin, antioxidants, lipid-based treatments | [104–107] |
Modulation of calcium signaling | IP3Rs, VDACs, MCU, NCLX, ATF6, PSEN2, PDK4, p53, | agonists/antagonists of key pathways, luteolin | [108–120] |
Targeting processes that regulate mitochondrial dynamics | DRP1, FIS1, MFN1, MFN2, OPA1, ATAD3A, AMPK | mdivi-1, dynasore, M1 compound, P110-TAT, trolox, dynarrestin, | [100,121–128] |
Funding: This work was supported by a British Heart Foundation (BHF) Programme Grant (RG/F/21/110050; award to Bernard D. Keavney), and by the Manchester Academic Health Science Centre (MAHSC), part of Health Innovation Manchester.
Institutional Review Board Statement: Not applicable.
Informed Consent Statement: Not applicable.
Data Availability Statement: Not applicable.
Conflicts of Interest: The authors declare no conflict of interest.

References
- Anderson, A.J.; Jackson, T.D.; Stroud, D.A.; et al. Mitochondria-hubs for regulating cellular biochemistry: emerging concepts and networks. Open Biol. 2019, 9, 190126, https://doi.org/10.1098/rsob.190126.
- Schwarz, D.S.; Blower, M.D. The endoplasmic reticulum: Structure, function and response to cellular signaling. Cell. Mol. Life Sci. 2016, 73, 79–94, https://doi.org/10.1007/s00018-015-2052-6.
- Braakman, I.; Hebert, D.N. Protein Folding in the Endoplasmic Reticulum. Cold Spring Harb. Perspect. Biol. 2013, 5, a013201, https://doi.org/10.1101/cshperspect.a013201.
- Sun, S.; Zhao, G.; Jia, M.; et al. Stay in touch with the endoplasmic reticulum. Sci. China Life Sci. 2024, 67, 230–257, https://doi.org/10.1007/s11427-023-2443-9.
- Yuan, M.; Gong, M.; He, J.; et al. IP3R1/GRP75/VDAC1 complex mediates endoplasmic reticulum stress-mitochondrial oxidative stress in diabetic atrial remodeling. Redox Biol. 2022, 52, 102289, https://doi.org/10.1016/j.redox.2022.102289.
- Filadi, R.; Leal, N.S.; Schreiner, B.; et al. TOM70 Sustains Cell Bioenergetics by Promoting IP3R3-Mediated ER to Mitochondria Ca(2+) Transfer. Curr Biol, 2018. 28, 369–382. https://doi.org/10.1016/j.cub.2017.12.047
- D’Eletto, M.; Rossin, F.; Occhigrossi, L.; et al. Transglutaminase Type 2 Regulates ER-Mitochondria Contact Sites by Interacting with GRP75. Cell Rep. 2018, 25, 3573‒3581, https://doi.org/10.1016/j.celrep.2018.11.094.
- Matsuzaki, H.; Fujimoto, T.; Tanaka, M.; et al. Tespa1 is a novel component of mitochondria-associated endoplasmic reticulum membranes and affects mitochondrial calcium flux. Biochem. Biophys. Res. Commun. 2013, 433, 322–326, https://doi.org/10.1016/j.bbrc.2013.02.099.
- Thoudam, T.; Ha, C.-M.; Leem, J.; et al. PDK4 Augments ER–Mitochondria Contact to Dampen Skeletal Muscle Insulin Signaling During Obesity. Diabetes 2018, 68, 571–586, https://doi.org/10.2337/db18-0363.
- Ilacqua, N.; Sánchez-Álvarez, M.; Bachmann, M.; et al. Protein Localization at Mitochondria-ER Contact Sites in Basal and Stress Conditions. Front. Cell Dev. Biol. 2017, 5, 107, https://doi.org/10.3389/fcell.2017.00107.
- Han, S.; Zhao, F.; Hsia, J.; et al. The role of Mfn2 in the structure and function of endoplasmic reticulum-mitochondrial tethering in vivo. J. Cell Sci. 2021, 134, jcs253443, https://doi.org/10.1242/jcs.253443.
- Naón, D.; Hernández-Alvarez, M.I.; Shinjo, S.; et al. Splice variants of mitofusin 2 shape the endoplasmic reticulum and tether it to mitochondria. Science 2023, 380, eadh9351, https://doi.org/10.1126/science.adh9351.
- Area-Gomez, E.; de Groof, A.J.C.; Boldogh, I.; et al. Presenilins Are Enriched in Endoplasmic Reticulum Membranes Associated with Mitochondria. Am. J. Pathol. 2009, 175, 1810–1816, doi:10.2353/ajpath.2009.090219.
- Contino, S.; Porporato, P.E.; Bird, M.; et al. Presenilin 2-Dependent Maintenance of Mitochondrial Oxidative Capacity and Morphology. Front. Physiol. 2017, 8, 796, https://doi.org/10.3389/fphys.2017.00796.
- Filadi, R.; Greotti, E.; Turacchio, G.; et al. Presenilin 2 Modulates Endoplasmic Reticulum-Mitochondria Coupling by Tuning the Antagonistic Effect of Mitofusin 2. Cell Rep. 2016, 15, 2226–2238, https://doi.org/10.1016/j.celrep.2016.05.013.
- Sugiura, A.; Nagashima, S.; Tokuyama, T.; et al. MITOL Regulates Endoplasmic Reticulum-Mitochondria Contacts via Mitofusin2. Mol. Cell 2013, 51, 20–34, https://doi.org/10.1016/j.molcel.2013.04.023.
- Yamano, K.; Kinefuchi, H.; Kojima, W. Mitochondrial lipid dynamics regulated by MITOL-mediated ubiquitination. J. Biochem. 2023, 175, 217–219, https://doi.org/10.1093/jb/mvad117.
- Wang, H.; Ju, D.; Kho, D.-H.; et al. The ubiquitin specific protease USP34 protects the ubiquitin ligase gp78 from proteasomal degradation. Biochem. Biophys. Res. Commun. 2019, 509, 348–353, https://doi.org/10.1016/j.bbrc.2018.12.141.
- De Vos, K.J.; Morotz, G.M.; Stoica, R.; et al. VAPB interacts with the mitochondrial protein PTPIP51 to regulate calcium homeostasis. Hum Mol Genet, 2012, 21, 1299–1311. doi: 10.1093/hmg/ddr559
- Mórotz, G.M.; Martín-Guerrero, S.M.; Markovinovic, A.; et al. The PTPIP51 coiled-coil domain is important in VAPB binding, formation of ER-mitochondria contacts and IP3 receptor delivery of Ca(2+) to mitochondria. Front Cell Dev Biol. 2022. 10, 920947. doi: 10.3389/fcell.2022.920947
- Iwasawa, R.; Mahul-Mellier, A.-L.; Datler, C.; et al. Fis1 and Bap31 bridge the mitochondria-ER interface to establish a platform for apoptosis induction. EMBO J. 2010, 30, 556–568, https://doi.org/10.1038/emboj.2010.346.
- Namba, T. BAP31 regulates mitochondrial function via interaction with Tom40 within ER-mitochondria contact sites. Sci. Adv. 2019, 5, eaaw1386, https://doi.org/10.1126/sciadv.aaw1386.
- Guillén-Samander, A.; Leonzino, M.; Hanna, IVM.G.; et al. VPS13D bridges the ER to mitochondria and peroxisomes via Miro. J. Cell Biol. 2021, 220, e202010004, https://doi.org/10.1083/jcb.202010004
- Hung, V.; Lam, S.S.; Udeshi, N.D., et al. Proteomic mapping of cytosol-facing outer mitochondrial and ER membranes in living human cells by proximity biotinylation. Elife 2017, 6, e24463, https://doi.org/10.7554/eLife.24463
- Doghman‐Bouguerra, M.; Granatiero, V.; Sbiera, S.; et al. FATE 1 antagonizes calcium‐ and drug‐induced apoptosis by uncoupling ER and mitochondria. Embo Rep. 2016, 17, 1264–1280, https://doi.org/10.15252/embr.201541504.
- Liao, H.-Y.; Liao, B.; Zhang, H.-H. CISD2 plays a role in age-related diseases and cancer. Biomed. Pharmacother. 2021, 138, 111472, https://doi.org/10.1016/j.biopha.2021.111472.
- Ilamathi, H.S.; Benhammouda, S.; Lounas, A.; et al. Contact sites between endoplasmic reticulum sheets and mitochondria regulate mitochondrial DNA replication and segregation. iScience 2023, 26, 107180, https://doi.org/10.1016/j.isci.2023.107180.
- Arguello, T.; Peralta, S.; Antonicka, H.; et al. ATAD3A has a scaffolding role regulating mitochondria inner membrane structure and protein assembly. Cell Rep. 2021, 37, 110139–110139, https://doi.org/10.1016/j.celrep.2021.110139.
- Wu, W.; Lin, C.; Wu, K.; et al. FUNDC 1 regulates mitochondrial dynamics at the ER –mitochondrial contact site under hypoxic conditions. EMBO J. 2016, 35, 1368–1384, https://doi.org/10.15252/embj.201593102.
- Harada, T.; Sada, R.; Osugi, Y.; et al. Palmitoylated CKAP4 regulates mitochondrial functions through an interaction with VDAC2 at ER-mitochondria contact sites. J. Cell Sci. 2020, 133, jcs249045, https://doi.org/10.1242/jcs.249045.
- Rühmkorf, A.; Harbauer, A.B. Role of Mitochondria–ER Contact Sites in Mitophagy. Biomolecules 2023, 13, 1198, https://doi.org/10.3390/biom13081198.
- Zhang, L.; Yan, F.; Li, L.; et al. New focuses on roles of communications between endoplasmic reticulum and mitochondria in identification of biomarkers and targets. Clin. Transl. Med. 2021, 11, e626, https://doi.org/10.1002/ctm2.626.
- Lim, D.; Dematteis, G.; Tapella, L.; et al. Ca2+ handling at the mitochondria-ER contact sites in neurodegeneration. Cell Calcium 2021, 98, 102453, https://doi.org/10.1016/j.ceca.2021.102453.
- Pichla, M.; Sneyers, F.; Stopa, K.B.; et al. Dynamic control of mitochondria-associated membranes by kinases and phosphatases in health and disease. Cell. Mol. Life Sci. 2021, 78, 6541–6556, https://doi.org/10.1007/s00018-021-03920-9.
- Morgado-Cáceres, P.; Liabeuf, G.; Calle, X.; et al. The aging of ER-mitochondria communication: A journey from undifferentiated to aged cells. Front Cell Dev Biol, 2022, 10, 946678, https://doi.org/10.3389/fcell.2022.946678
- Bergami, M.; Motori, E. Reweaving the Fabric of Mitochondrial Contact Sites in Astrocytes. Front. Cell Dev. Biol. 2020, 8, 592651, https://doi.org/10.3389/fcell.2020.592651.
- Yu, F.; Courjaret, R.; Assaf, L.; et al. Mitochondria-ER contact sites expand during mitosis. iScience 2024, 27, 109379, https://doi.org/10.1016/j.isci.2024.109379.
- De Strooper, B.; Scorrano, L. Close encounter: mitochondria, endoplasmic reticulum and Alzheimer’s disease. EMBO J. 2012, 31, 4095–4097, https://doi.org/10.1038/emboj.2012.279.
- Li, Z.; Cao, Y.; Pei, H.; et al. The contribution of mitochondria-associated endoplasmic reticulum membranes (MAMs) dysfunction in Alzheimer’s disease and the potential countermeasure. Front. Neurosci. 2023, 17, 1158204, https://doi.org/10.3389/fnins.2023.1158204.
- Wilson, E.L.; Metzakopian, E. ER-mitochondria contact sites in neurodegeneration: genetic screening approaches to investigate novel disease mechanisms. Cell Death Differ 2021, 28, 1804–1821. doi: 10.1038/s41418-020-00705-8
- Dentoni, G.; Castro-Aldrete, L.; Naia, L.; et al. The Potential of Small Molecules to Modulate the Mitochondria–Endoplasmic Reticulum Interplay in Alzheimer’s Disease. Front. Cell Dev. Biol. 2022, 10, 920228, https://doi.org/10.3389/fcell.2022.920228.
- Jagtap, Y.A.; Kumar, P.; Kinger, S.; et al. Disturb mitochondrial associated proteostasis: Neurodegeneration and imperfect ageing. Front Cell Dev Biol. 2023, 11, 1146564. https://doi.org/10.3389/fcell.2023.1146564
- Area-Gomez, E.; Schon, E.A. Towards a Unitary Hypothesis of Alzheimer’s Disease Pathogenesis. J. Alzheimer’s Dis. 2024, 98, 1243‒1275, https://doi.org/10.3233/jad-231318.
- Xu, L.; Wang, X.; Tong, C. Endoplasmic Reticulum–Mitochondria Contact Sites and Neurodegeneration. Front. Cell Dev. Biol. 2020, 8, 428, https://doi.org/10.3389/fcell.2020.00428.
- Han, J.; Park, H.; Maharana, C.; et al. Alzheimer’s disease-causing presenilin-1 mutations have deleterious effects on mitochondrial function. Theranostics 2021, 11, 8855–8873. doi: 10.7150/thno.59776
- Barodia, S.K.; Prabhakaran, K.; Karunakaran, S.; et al. Editorial: Mitochondria and Endoplasmic Reticulum Dysfunction in Parkinson’s Disease. Front Neurosci, 2019, 13, 1171, https://doi.org/10.3389/fnins.2019.01171.
- Xu, J.; Minobe, E.; Kameyama, M. Ca2+ Dyshomeostasis Links Risk Factors to Neurodegeneration in Parkinson’s Disease. Front. Cell. Neurosci. 2022, 16, 867385, https://doi.org/10.3389/fncel.2022.867385.
- Guillén-Samander, A.; De Camilli, P. Endoplasmic Reticulum Membrane Contact Sites, Lipid Transport, and Neurodegeneration. Cold Spring Harb Perspect Biol. 2023, 15, a041257. doi: 10.1101/cshperspect.a041257
- Prasuhn, J.; Davis, R.L.; Kumar, K.R. Targeting Mitochondrial Impairment in Parkinson’s Disease: Challenges and Opportunities. Front Cell Dev Biol. 2020, 8, 615461, https://doi.org/10.3389/fcell.2020.615461.
- Maity, S.; Komal, P.; Kumar, V.; et al. Impact of ER Stress and ER-Mitochondrial Crosstalk in Huntington’s Disease. Int. J. Mol. Sci. 2022, 23, 780, https://doi.org/10.3390/ijms23020780.
- Johri, A.; Chandra, A.; Connection Lost, MAM: Errors in ER–Mitochondria Connections in Neurodegenerative Diseases. Brain Sci, 2021, 11, 1437, https://doi.org/10.3390/brainsci11111437.
- Cherubini, M.; Lopez-Molina, L.; Gines, S. Mitochondrial fission in Huntington’s disease mouse striatum disrupts ER-mitochondria contacts leading to disturbances in Ca(2+) efflux and Reactive Oxygen Species (ROS) homeostasis. Neurobiol Dis. 2020, 136, 104741, https://doi.org/10.1016/j.nbd.2020.104741.
- Bernal, A.F.; Mota, N.; Pamplona, R.; et al. Hakuna MAM-Tata: Investigating the role of mitochondrial-associated membranes in ALS. Biochim. et Biophys. Acta (BBA) - Mol. Basis Dis. 2023, 1869, 166716, https://doi.org/10.1016/j.bbadis.2023.166716.
- Martín-Guerrero, S.M.; Markovinovic, A.; Mórotz, G.M.; et al. Targeting ER-Mitochondria Signaling as a Therapeutic Target for Frontotemporal Dementia and Related Amyotrophic Lateral Sclerosis. Front. Cell Dev. Biol. 2022, 10, 915931, https://doi.org/10.3389/fcell.2022.915931.
- Chen, J.; Bassot, A.; Giuliani, F.; et al. Amyotrophic Lateral Sclerosis (ALS): Stressed by Dysfunctional Mitochondria-Endoplasmic Reticulum Contacts (MERCs). Cells 2021, 10, 1789, https://doi.org/10.3390/cells10071789.
- Hartopp, N.; Markovinovic, A.; Miller, C.C.J.; et al., Insight into endoplasmic reticulum-mitochondria contacts in human amyotrophic lateral sclerosis. Neural. Regen. Res. 2024, 19, 1407‒1408. doi: 10.4103/1673-5374.387988
- Wilson, E.L.; Yu, Y.; Leal, N.S.; et al. Genome-wide CRISPR/Cas9 screen shows that loss of GET4 increases mitochondria-endoplasmic reticulum contact sites and is neuroprotective. Cell Death Dis. 2024, 15, 1–16, https://doi.org/10.1038/s41419-024-06568-y.
- Yang, S.; Zhou, R.; Zhang, C.; et al. Mitochondria-Associated Endoplasmic Reticulum Membranes in the Pathogenesis of Type 2 Diabetes Mellitus. Front. Cell Dev. Biol. 2020, 8, 571554, https://doi.org/10.3389/fcell.2020.571554.
- Cheng, H.; Gang, X.; He, G.; et al. The Molecular Mechanisms Underlying Mitochondria-Associated Endoplasmic Reticulum Membrane-Induced Insulin Resistance. Front. Endocrinol. 2020, 11, 592129 https://doi.org/10.3389/fendo.2020.592129.
- Thivolet, C.; Vial, G.; Cassel, R.; et al. Reduction of endoplasmic reticulum- mitochondria interactions in beta cells from patients with type 2 diabetes. PLOS ONE 2017, 12, e0182027–e0182027, https://doi.org/10.1371/journal.pone.0182027.
- Rieusset, J. The role of endoplasmic reticulum-mitochondria contact sites in the control of glucose homeostasis: an update. Cell Death Dis. 2018, 9, 1–12, https://doi.org/10.1038/s41419-018-0416-1.
- Dingreville, F.; Panthu, B.; Thivolet, C.; et al. Differential Effect of Glucose on ER-Mitochondria Ca(2+) Exchange Participates in Insulin Secretion and Glucotoxicity-Mediated Dysfunction of beta-Cells. Diabetes, 2019, 68, 1778–1794. doi: 10.2337/db18-1112
- Song, H.; Zhang, X.; Wang, J.; et al. The regulatory role of adipocyte mitochondrial homeostasis in metabolism-related diseases. Front. Physiol. 2023, 14, 1261204, https://doi.org/10.3389/fphys.2023.1261204.
- Xia, W.; Veeragandham, P.; Cao, Y.; et al. Obesity causes mitochondrial fragmentation and dysfunction in white adipocytes due to RalA activation. Nat. Metab. 2024, 6, 273–289, https://doi.org/10.1038/s42255-024-00978-0.
- Beaulant, A.; Dia, M.; Pillot, B.; et al. Endoplasmic reticulum-mitochondria miscommunication is an early and causal trigger of hepatic insulin resistance and steatosis. J. Hepatol. 2022, 77, 710–722, https://doi.org/10.1016/j.jhep.2022.03.017.
- de Almeida, M.E.; Ørtenblad, N.; Petersen, M.H.; et al. Acute exercise increases the contact between lipid droplets and mitochondria independently of obesity and type 2 diabetes. J. Physiol, 2023, 601, 1797–1815. doi: 10.1113/JP284386
- Wang, J.; He, W.; Tsai, P.-J.; et al. Mutual interaction between endoplasmic reticulum and mitochondria in nonalcoholic fatty liver disease. Lipids Heal. Dis. 2020, 19, 1–19, https://doi.org/10.1186/s12944-020-01210-0.
- Barbier-Torres, L.; Fortner, K.A.; Iruzubieta, P.; et al. Silencing hepatic MCJ attenuates non-alcoholic fatty liver disease (NAFLD) by increasing mitochondrial fatty acid oxidation. Nat. Commun. 2020, 11, 1–15, https://doi.org/10.1038/s41467-020-16991-2.
- Dabravolski, S.A.; Bezsonov, E.E.; Orekhov, A.N. The role of mitochondria dysfunction and hepatic senescence in NAFLD development and progression. Biomed. Pharmacother. 2021, 142, 112041, https://doi.org/10.1016/j.biopha.2021.112041.
- Jin, C.; Felli, E.; Lange, N.F.; et al. Endoplasmic Reticulum and Mitochondria Contacts Correlate with the Presence and Severity of NASH in Humans. Int. J. Mol. Sci. 2022, 23, 8348, https://doi.org/10.3390/ijms23158348.
- Myint, M.; Oppedisano, F.; De Giorgi, V.; et al. Inflammatory signaling in NASH driven by hepatocyte mitochondrial dysfunctions. J. Transl. Med. 2023, 21, 1–16, https://doi.org/10.1186/s12967-023-04627-0.
- Hernández-Alvarez, M.I.; Sebastián, D.; Vives, S.; et al. Deficient Endoplasmic Reticulum-Mitochondrial Phosphatidylserine Transfer Causes Liver Disease. Cell 2019, 177, 881–895, https://doi.org/10.1016/j.cell.2019.04.010.
- Forte, M.; Schirone, L.; Ameri, P.; et al. The role of mitochondrial dynamics in cardiovascular diseases. Br. J. Pharmacol. 2020, 178, 2060–2076, https://doi.org/10.1111/bph.15068.
- Gao, P.; Yan, Z.; Zhu, Z. Mitochondria-Associated Endoplasmic Reticulum Membranes in Cardiovascular Diseases. Front. Cell Dev. Biol. 2020, 8, 604240, https://doi.org/10.3389/fcell.2020.604240.
- Li, J.; Zhang, D.; Brundel, B.J.; et al. Imbalance of ER and Mitochondria Interactions: Prelude to Cardiac Ageing and Disease? Cells 2019, 8, 1617, https://doi.org/10.3390/cells8121617.
- Moro, L. Mitochondria at the Crossroads of Physiology and Pathology. J. Clin. Med. 2020, 9, 1971, https://doi.org/10.3390/jcm9061971.
- Peoples, J.N.; Saraf, A.; Ghazal, N.; et al. Mitochondrial dysfunction and oxidative stress in heart disease. Exp. Mol. Med. 2019, 51, 1–13, https://doi.org/10.1038/s12276-019-0355-7.
- Maamoun, H.; Abdelsalam, S.S.; Zeidan, A.; et al. Endoplasmic Reticulum Stress: A Critical Molecular Driver of Endothelial Dysfunction and Cardiovascular Disturbances Associated with Diabetes. Int. J. Mol. Sci. 2019, 20, 1658, https://doi.org/10.3390/ijms20071658.
- Peruzzo, R.; Costa, R.; Bachmann, M.; et al. Mitochondrial Metabolism, Contact Sites and Cellular Calcium Signaling: Implications for Tumorigenesis. Cancers 2020, 12, 2574, https://doi.org/10.3390/cancers12092574.
- Bustos, G.; Ahumada-Castro, U.; Silva-Pavez, E.; et al. The ER-mitochondria Ca(2+) signaling in cancer progression: Fueling the monster. Int. Rev. Cell Mol. Biol, 2021, 363, 49–121. doi: 10.1016/bs.ircmb.2021.03.006
- Reyes-Castellanos, G.; Hadi, N.A.; Carrier, A. Autophagy Contributes to Metabolic Reprogramming and Therapeutic Resistance in Pancreatic Tumors. Cells 2022, 11, 426, https://doi.org/10.3390/cells11030426.
- Themistocleous, S.; Christodoulou, P.; Kyriakou, T.-C.; et al. Key genes expressed in mitochondria-endoplasmic reticulum contact sites in cancer (Review). Oncol. Rep. 2023, 49, 1–14, https://doi.org/10.3892/or.2023.8514.
- Nobili, A.; Krashia, P.; D’Amelio, M. Cisd2: a promising new target in Alzheimer’s disease(dagger). J. Pathol. 2020, 251, 113–116. doi: 10.1002/path.5436
- Yeh, C.-H.; Chou, Y.-J.; Kao, C.-H.; et al. Mitochondria and Calcium Homeostasis: Cisd2 as a Big Player in Cardiac Ageing. Int. J. Mol. Sci. 2020, 21, 9238, https://doi.org/10.3390/ijms21239238.
- Li, S.X.; Li, J.; Dong, L.W.; et al. Cytoskeleton-Associated Protein 4, a Promising Biomarker for Tumor Diagnosis and Therapy. Fron. Mole. Biosci., 2021, 7, 552056. doi: 10.3389/fmolb.2020.552056
- Simoes, I.C.M.; Morciano, G.; Lebiedzinska-Arciszewska, M.; et al. The mystery of mitochondria-ER contact sites in physiology and pathology: A cancer perspective. Biochim. et Biophys. Acta (BBA) - Mol. Basis Dis. 2020, 1866, 165834, https://doi.org/10.1016/j.bbadis.2020.165834.
- Grespi, F.; Vianello, C.; Cagnin, S.; et al. The Interplay of Microtubules with Mitochondria–ER Contact Sites (MERCs) in Glioblastoma. Biomolecules 2022, 12, 567, https://doi.org/10.3390/biom12040567.
- Sammeta, S.S.; Banarase, T.A.; Rahangdale, S.R.; et al. Molecular understanding of ER-MT communication dysfunction during neurodegeneration. Mitochondrion 2023, 72, 59–71, https://doi.org/10.1016/j.mito.2023.07.005.
- Hajimahdi, Z. Small Molecules as Protein-Protein Interaction Inhibitors. Iran, J. Pharm. Res, 2016, 15, 1–2.
- Hartopp, N.; Lau, D.H.W.; Martin-Guerrero, S.M.; et al. Disruption of the VAPB-PTPIP51 ER-mitochondria tethering proteins in post-mortem human amyotrophic lateral sclerosis. Front. Cell Dev. Biol. 2022, 10, 950767, https://doi.org/10.3389/fcell.2022.950767.
- Li, M.; Zhang, Y.; Yu, G.; et al. Mitochondria‐associated endoplasmic reticulum membranes tethering protein VAPB‐PTPIP51 protects against ischemic stroke through inhibiting the activation of autophagy. CNS Neurosci. Ther. 2024, 30, e14707, https://doi.org/10.1111/cns.14707.
- Zhang, C.; Liu, B.; Sheng, J.; et al. Potential targets for the treatment of MI: GRP75-mediated Ca2+ transfer in MAM. Eur. J. Pharmacol. 2024, 971, 176530, https://doi.org/10.1016/j.ejphar.2024.176530.
- Wu, H.; Wang, Y.; Li, W.; et al. Deficiency of mitophagy receptor FUNDC1 impairs mitochondrial quality and aggravates dietary-induced obesity and metabolic syndrome. Autophagy 2019, 15, 1882–1898, https://doi.org/10.1080/15548627.2019.1596482.
- Zhang, T.; Kho, D.H.; Wang, Y.; et al. Gp78, an E3 Ubiquitin Ligase Acts as a Gatekeeper Suppressing Nonalcoholic Steatohepatitis (NASH) and Liver Cancer. PLOS ONE 2015, 10, e0118448, https://doi.org/10.1371/journal.pone.0118448.
- Zhao, H.; Song, G.; Zhu, H.; et al. Pharmacological Effects of Urolithin A and Its Role in Muscle Health and Performance: Current Knowledge and Prospects. Nutrients 2023, 15, 4441, https://doi.org/10.3390/nu15204441.
- Mazarakis, N.; Snibson, K.; Licciardi, P.V.; et al. The potential use of L-sulforaphane for the treatment of chronic inflammatory diseases: A review of the clinical evidence. Clin. Nutr. 2020, 39, 664–675, https://doi.org/10.1016/j.clnu.2019.03.022.
- Rong, Y.P.; Bultynck, G.; Aromolaran, A.S.; et al., The BH4 domain of Bcl-2 inhibits ER calcium release and apoptosis by binding the regulatory and coupling domain of the IP3 receptor. Proc. Natl. Acad. Sci. 2009, 106, 14397‒14402. doi: 10.1073/pnas.0907555106
- Janer, A.; Morris, J.L.; Krols, M.; et al. ESYT1 tethers the ER to mitochondria and is required for mitochondrial lipid and calcium homeostasis. Life Sci. Alliance 2023, 7, e202302335, https://doi.org/10.26508/lsa.202302335.
- Huo, Z.; Gu, J.; He, T. Apelin-13 reduces high glucose-induced mitochondrial dysfunction in cochlear hair cells by inhibiting endoplasmic reticulum stress. Exp. Ther. Med. 2024, 27, 1–8, https://doi.org/10.3892/etm.2024.12515.
- Su, Z.D.Z.; Li, C.Q.; Wang, H.W.; et al. Inhibition of DRP1-dependent mitochondrial fission by Mdivi-1 alleviates atherosclerosis through the modulation of M1 polarization. J. Transl. Med. 2023, 21, 427. doi: 10.1186/s12967-023-04270-9
- Li, F.; Aljahdali, I.A.M.; Ling, X. Molecular Glues: Capable Protein-Binding Small Molecules That Can Change Protein–Protein Interactions and Interactomes for the Potential Treatment of Human Cancer and Neurodegenerative Diseases. Int. J. Mol. Sci. 2022, 23, 6206, https://doi.org/10.3390/ijms23116206.
- Thai, P.N.; Seidlmayer, L.K.; Miller, C.; et al. Mitochondrial Quality Control in Aging and Heart Failure: Influence of Ketone Bodies and Mitofusin-Stabilizing Peptides. Front. Physiol. 2019, 10, 382, https://doi.org/10.3389/fphys.2019.00382.
- Oh, J.G.; Kim, J.; Jang, S.P.; et al. Decoy peptides targeted to protein phosphatase 1 inhibit dephosphorylation of phospholamban in cardiomyocytes. J. Mol. Cell. Cardiol. 2013, 56, 63–71. doi: 10.1016/j.yjmcc.2012.12.005
- Peretti, D.; Kim, S.; Tufi, R.; et al. Lipid Transfer Proteins and Membrane Contact Sites in Human Cancer. Front. Cell Dev. Biol. 2020, 7, 371, https://doi.org/10.3389/fcell.2019.00371.
- Xia, Y.; Zhang, Y.; Sun, Y.; et al. CCDC127 regulates lipid droplet homeostasis by enhancing mitochondria-ER contacts. Biochem. Biophys. Res. Commun. 2023, 683, 149116, https://doi.org/10.1016/j.bbrc.2023.10.048.
- Muallem, S.; Chung, W.Y.; Jha, A.; et al. Lipids at membrane contact sites: cell signaling and ion transport. Embo Rep. 2017, 18, 1893–1904, https://doi.org/10.15252/embr.201744331.
- Lin, H.; Guo, X.; Liu, J.; et al. Ethanol‐Induced Hepatic Ferroptosis Is Mediated by PERK‐Dependent MAMs Formation: Preventive Role of Quercetin. Mol. Nutr. Food Res. 2024, 68, e2300343, https://doi.org/10.1002/mnfr.202300343.
- Kerkhofs, M.; Bultynck, G.; Vervliet, T.; et al. Therapeutic implications of novel peptides targeting ER–mitochondria Ca2+-flux systems. Drug Discov. Today 2019, 24, 1092–1103, https://doi.org/10.1016/j.drudis.2019.03.020.
- Gambardella, J.; Morelli, M.B.; Wang, X.; et al. The discovery and development of IP3 receptor modulators: an update. Expert Opin. Drug Discov. 2021, 16, 709–718, https://doi.org/10.1080/17460441.2021.1858792.
- Zakyrjanova, G.F.; Gilmutdinov, A.; Tsentsevitsky, A.N.; et al. Olesoxime, a cholesterol-like neuroprotectant restrains synaptic vesicle exocytosis in the mice motor nerve terminals: Possible role of VDACs. Biochim. et Biophys. Acta (BBA) - Mol. Cell Biol. Lipids 2020, 1865, 158739, https://doi.org/10.1016/j.bbalip.2020.158739.
- Chen, W.; Shen, Z.; Dong, W.; et al. Polygonatum sibiricum polysaccharide ameliorates skeletal muscle aging via mitochondria-associated membrane-mediated calcium homeostasis regulation. Phytomedicine 2024, 129, 155567. doi: 10.1016/j.phymed.2024.155567
- Wang, J.; Jiang, J.; Hu, H.; et al. MCU complex: Exploring emerging targets and mechanisms of mitochondrial physiology and pathology. J. Adv. Res. 2024, https://doi.org/10.1016/j.jare.2024.02.013.
- Wang, S.; Hu, B.; Ding, Z.; et al. ATF6 safeguards organelle homeostasis and cellular aging in human mesenchymal stem cells. Cell Discov. 2018, 4, 2, https://doi.org/10.1038/s41421-017-0003-0.
- Huang, J.; Wan, L.; Lu, H.; et al. High expression of active ATF6 aggravates endoplasmic reticulum stress-induced vascular endothelial cell apoptosis through the mitochondrial apoptotic pathway. Mol. Med. Rep. 2018, 17, 6483–6489, https://doi.org/10.3892/mmr.2018.8658.
- Burkewitz, K.; Feng, G.; Dutta, S.; et al. Atf-6 Regulates Lifespan through ER-Mitochondrial Calcium Homeostasis. Cell Rep. 2020, 32, 108125. doi: 10.1016/j.celrep.2020.108125
- Rossi, A.; Galla, L.; Gomiero, C.; et al. Calcium Signaling and Mitochondrial Function in Presenilin 2 Knock-Out Mice: Looking for Any Loss-of-Function Phenotype Related to Alzheimer’s Disease. Cells 2021, 10, 204, https://doi.org/10.3390/cells10020204.
- Thoudam, T.; Chanda, D.; Lee, J.Y.; et al. Enhanced Ca2+-channeling complex formation at the ER-mitochondria interface underlies the pathogenesis of alcohol-associated liver disease. Nat. Commun. 2023, 14, 1–18, https://doi.org/10.1038/s41467-023-37214-4.
- Giorgi, C.; Bonora, M.; Sorrentino, G.; et al. p53 at the endoplasmic reticulum regulates apoptosis in a Ca2+-dependent manner. Proc. Natl. Acad. Sci. 2015, 112, 1779–1784, https://doi.org/10.1073/pnas.1410723112.
- Naia, L.; Pinho, C.M.; Dentoni, G.; et al. Neuronal cell-based high-throughput screen for enhancers of mitochondrial function reveals luteolin as a modulator of mitochondria-endoplasmic reticulum coupling. BMC Biol. 2021, 19, 1–21, https://doi.org/10.1186/s12915-021-00979-5.
- Ntalouka, F.; Tsirivakou, A. Luteolin: A promising natural agent in management of pain in chronic conditions. Front. Pain Res. 2023, 4, 1114428, https://doi.org/10.3389/fpain.2023.1114428.
- Chen, W.; Zhao, H.; Li, Y. Mitochondrial dynamics in health and disease: mechanisms and potential targets. Signal Transduct. Target. Ther. 2023, 8, 1–25, https://doi.org/10.1038/s41392-023-01547-9.
- Rakhmatullina, D.; Mazina, A.; Ponomareva, A.; et al. Mdivi-1 Induced Mitochondrial Fusion as a Potential Mechanism to Enhance Stress Tolerance in Wheat. Life 2022, 12, 1386, https://doi.org/10.3390/life12091386.
- Muñoz, J.P.; Basei, F.L.; Rojas, M.L.; et al. Mechanisms of Modulation of Mitochondrial Architecture. Biomolecules 2023, 13, 1225, https://doi.org/10.3390/biom13081225.
- Chen, L.; Li, Y.; Zambidis, A.; et al. ATAD3A: A Key Regulator of Mitochondria-Associated Diseases. Int. J. Mol. Sci. 2023, 24, 12511, https://doi.org/10.3390/ijms241512511.
- de Marañón, A.M.; Díaz-Pozo, P.; Canet, F.; et al. Metformin modulates mitochondrial function and mitophagy in peripheral blood mononuclear cells from type 2 diabetic patients. Redox. Biol. 2022, 53, 102342. doi: 10.1016/j.redox.2022.102342
- Distelmaier, F.; Visch, H.-J.; Smeitink, J.A.M.; et al. The antioxidant Trolox restores mitochondrial membrane potential and Ca2+-stimulated ATP production in human complex I deficiency. J. Mol. Med. 2009, 87, 515–522, https://doi.org/10.1007/s00109-009-0452-5.
- Höing, S.; Yeh, T.-Y.; Baumann, M.; et al. Dynarrestin, a Novel Inhibitor of Cytoplasmic Dynein. Cell Chem. Biol. 2018, 25, 357–369, https://doi.org/10.1016/j.chembiol.2017.12.014.
- Rout, S.K.; Priya, V.; Setia, A.; et al. Mitochondrial targeting theranostic nanomedicine and molecular biomarkers for efficient cancer diagnosis and therapy. Biomed Pharmacother 2022, 153, 113451. doi: 10.1016/j.biopha.2022.113451
- Bano, I.; Butt, U.D.; Mohsan, S.A.H. New challenges in drug discovery. In Novel Platforms for Drug Delivery Applications. 2023, pp. 619–643. https://doi.org/10.1016/B978-0-323-91376-8.00021-5
- Ezike, T.C.; Okpala, U.S.; Onoja, U.L.; et al. Advances in drug delivery systems, challenges and future directions. Heliyon 2023, 9, e17488. doi: 10.1016/j.heliyon.2023.e17488
- Aoyama-Ishiwatari, S.; Hirabayashi, Y. Endoplasmic Reticulum–Mitochondria Contact Sites—Emerging Intracellular Signaling Hubs. Front. Cell Dev. Biol. 2021, 9, 653828, https://doi.org/10.3389/fcell.2021.653828.
- Dhanasekaran, S.; Venugopal, D.; Al-Dayan, N.; et al. Emerging insights into mitochondria-specific targeting and drug delivering strategies: Recent milestones and therapeutic implications. Saudi, J. Biol. Sci. 2020, 27, 3581–3592, https://doi.org/10.1016/j.sjbs.2020.07.030.
- Sulaimon, L.A.; Afolabi, L.O.; Adisa, R.A.; et al. Pharmacological significance of MitoQ in ameliorating mitochondria-related diseases. Adv. Redox Res. 2022, 5, 100037, https://doi.org/10.1016/j.arres.2022.100037.
- Milane, L.S.; Dolare, S.; Ren, G.; et al. Combination Organelle Mitochondrial Endoplasmic Reticulum Therapy (COMET) for Multidrug Resistant Breast Cancer. J. Control. Release 2023, 363, 435–451, https://doi.org/10.1016/j.jconrel.2023.09.023.
- Wang, W.; Zhang, Y.; Wang, Z.; et al. A Native Drug-Free Macromolecular Therapeutic to Trigger Mutual Reinforcing of Endoplasmic Reticulum Stress and Mitochondrial Dysfunction for Cancer Treatment. ACS Nano 2023, 17, 11023–11038, https://doi.org/10.1021/acsnano.3c03450.
- Singh, A.; Faccenda, D.; Campanella, M. Pharmacological advances in mitochondrial therapy. EBioMedicine 2021, 65, 103244, https://doi.org/10.1016/j.ebiom.2021.103244.